Stars, although they’re incredibly stable over very long timescales, simply cannot live forever. No matter what their composition, mass, temperature, or other properties may be, every star will eventually run out of the nuclear fuel that powers it. For all but the most massive stars in the Universe, each one will leave behind a remnant core: a white dwarf. Most Sun-like stars will leave behind white dwarfs that are mostly made of carbon-and-oxygen, with some helium, neon, magnesium, and silicon alongside them, and these remnants will continue shining brightly for trillions of years to come.
But unlike the Sun, which is all by itself in the Solar System, approximately half of all stars have at least one companion, and are members of multi-star systems. As time goes by, all stars burn through their fuel, but the most massive stars burn though their fuel the fastest. As a result, they end their lives and become dense remnants first, allowing them to begin stealing mass from their remaining companions.
When a white dwarf steals enough mass, they go nova. But these nova aren’t what we originally thought they were, and the discovery of the fastest nova ever, V1674 Hercules, teaches us more than we could’ve imagined just a few short years ago. A catastrophe is soon coming for this stellar remnant. Here’s what we know about it.
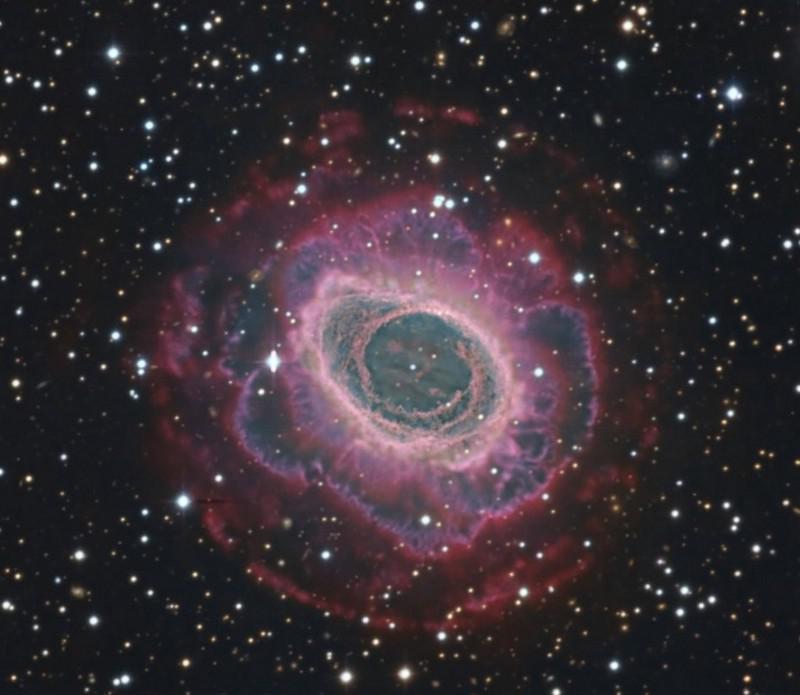
Once a star runs out of the last of its fuel in its core, its days as a full-fledged star are over. While the most massive stars will have their cores collapse in a catastrophic supernova explosion, most stars will blow off their outer layers gently, leaving the remnant core to contract down, trapping the star’s heat inside, and transitioning into a white dwarf star.
There’s a limit to how massive a white dwarf can be: about 1.4 times the mass of our Sun. Below that mass threshold, electrons and atomic nuclei in the white dwarf’s core will experience a quantum mechanical pressure from the Pauli Exclusion Principle that holds them up against gravitational collapse. But if the mass ever rises above that threshold, the white dwarf’s core will collapse, creating a runaway nuclear reaction and destroying it entirely in a type Ia supernova explosion.
Perhaps paradoxically, the more massive a white dwarf is, the smaller, denser, and hotter it will be. The more massive it is, the closer it is to that critical threshold, where it will experience a catastrophic cataclysm and self-destruct. But, unless it’s going to collide with another white dwarf, its journey toward this destruction is slow and gradual, driven by the accretion of material from a companion star.
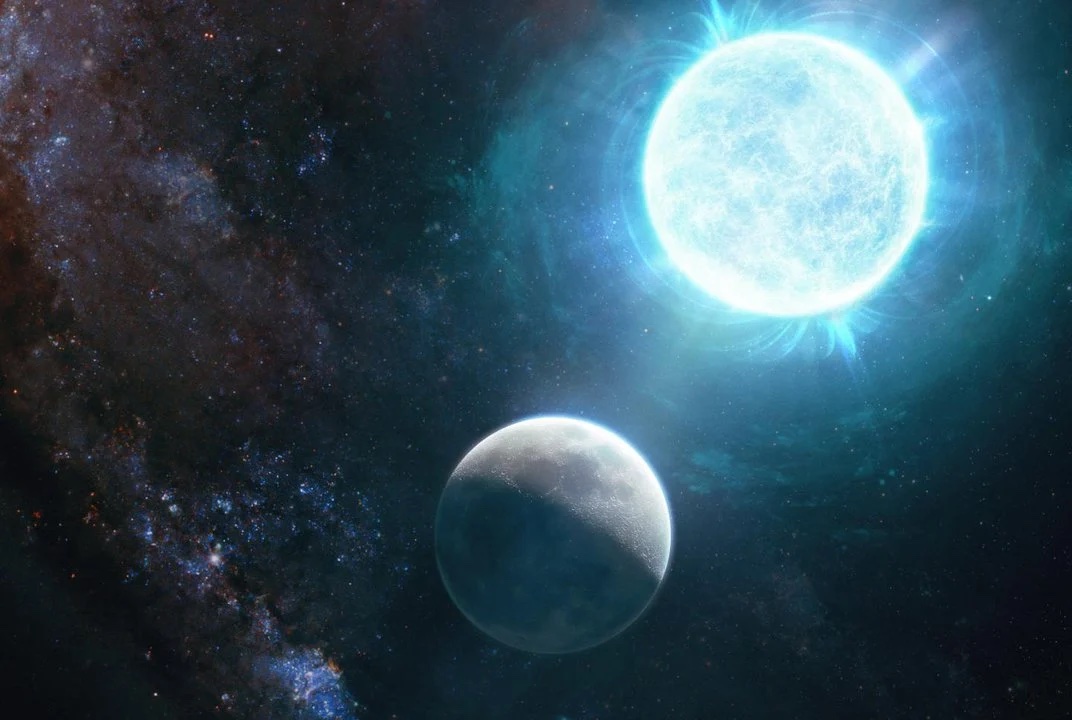
This is where, originally, the idea of a nova came from. A nova is a burst of brightness that greatly enhances a white dwarf’s luminosity, and then gradually falls back down to its original brightness over time. It’s known to be caused by the accumulation of matter from a companion star onto a white dwarf, and when enough mass accumulates to trigger a thermonuclear reaction, a nova is the result.
Although the relatively more spectacular phenomena of either a supernova or a kilonova are often all the rage, the nova has one fundamental advantage: it doesn’t destroy either of the progenitor objects that give rise to it. Instead, a nova simply represents an outburst of activity and a release of energy, symbolic of bringing a white dwarf closer and closer to the critical threshold where it can no longer hold itself up against gravitational collapse.
Over long enough timescales, just as you’d expect, these novae can recharge. As long as you’re continuously accreting matter from a companion star, each “flare” of a nova — even though it might take centuries or millennia for them to typically recur — so long as neither the white dwarf nor the companion star get disrupted, will be both preceded and followed by another one.
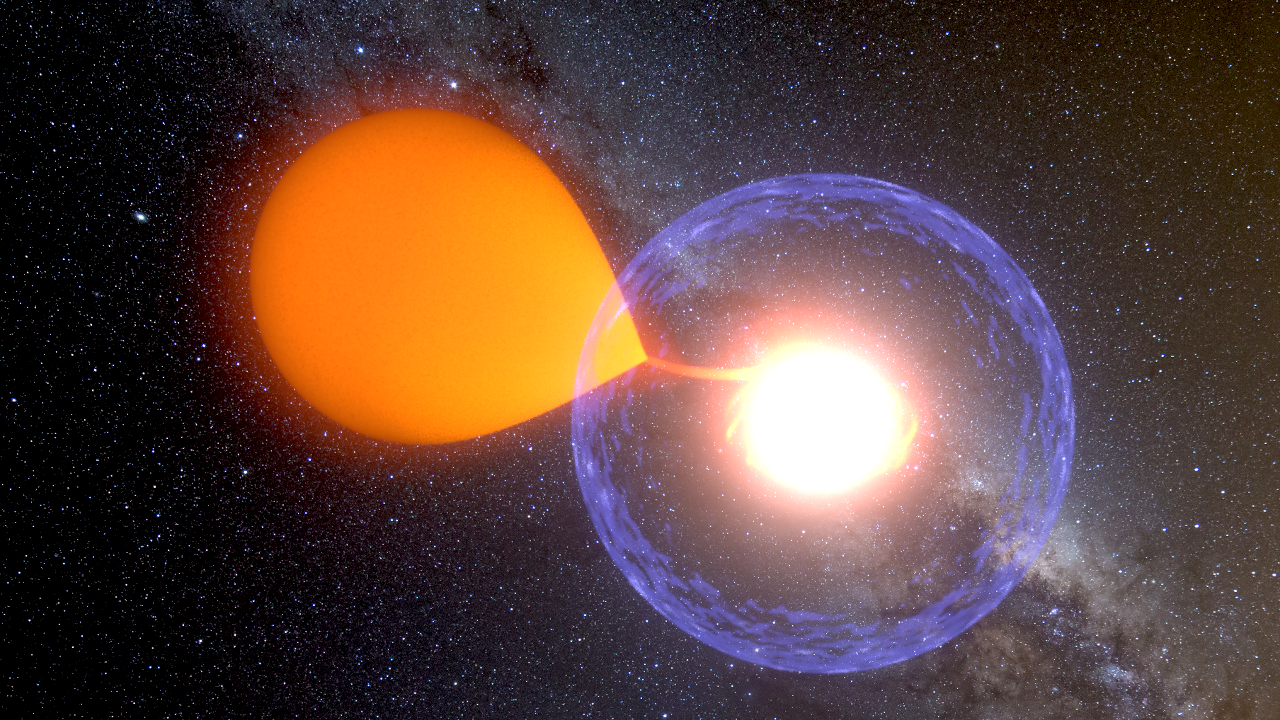
Originally, astronomers had a simple, naive, and incorrect picture of how these nova events occurred.
- The dense, compact stellar remnant — the white dwarf — begins to siphon off the most loosely held matter from the outer layers of the (closest) companion star.
- That material — mostly hydrogen gas — accumulates on the outside of the white dwarf.
- As the accumulated mass builds up, the surface material gets denser and hotter, and at some point, a nuclear reaction occurs, similar to how a nuclear “shell” of lighter material fuses together around the core of a giant star.
- And then, the nuclear reaction propagates throughout the remainder of the star’s surface material, triggering what we see as a nova, adding slightly to the mass of the white dwarf, all while blowing the surface material away.
Some of the novae that are out there have tight, close-orbiting binary companions, and those are the novae that recharge and go off again the fastest: the recurrent novae. While most novae are expected to take somewhere on the order of millennia to recharge, some take mere centuries or even just decades to recharge. The fastest repeated novae ever observed can experience just 8 or 9 years between recurrences.
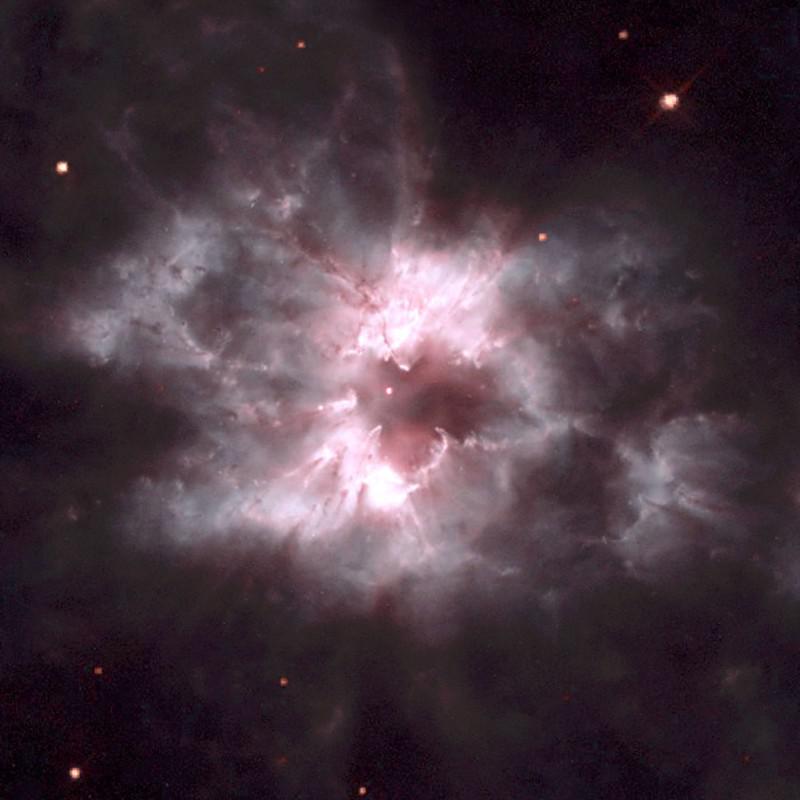
But this picture that astronomers initially painted for themselves, above, is not quite correct, and there are two clues that brought us there.
- One of the greatest clues we’ve gotten comes from simply watching how the light from a nova, once it reaches its peak brightness, fades over time. When astronomers talk about the “speed” of a nova — whether it’s fast or slow — they’re not talking, as you might expect, about the amount of time it takes a white dwarf to recharge and trigger the next nova after its previous one. (After all, only about 10 recurrent novae are known!) Instead, they’re talking about the amount of time it takes the nova to fade away. The ones that fade more quickly are “fast;” the ones that fade more slowly are “slow.”
- The second clue we can add in comes from observing the binary companion stars that are orbiting the white dwarfs that are generating the novae in question. Most of the novae that we’ve seen come from white dwarfs that have binary companions in very close, tight orbits; that’s no surprise, as a more easily-siphoned fuel source is more likely to lead to an observed nova. What we can also learn, however, is what the mass of the white dwarf star is from the gravitational, orbital properties of the companion star.
We can then look at the other properties of each nova in the context of the nova’s speed and the white dwarf’s mass.
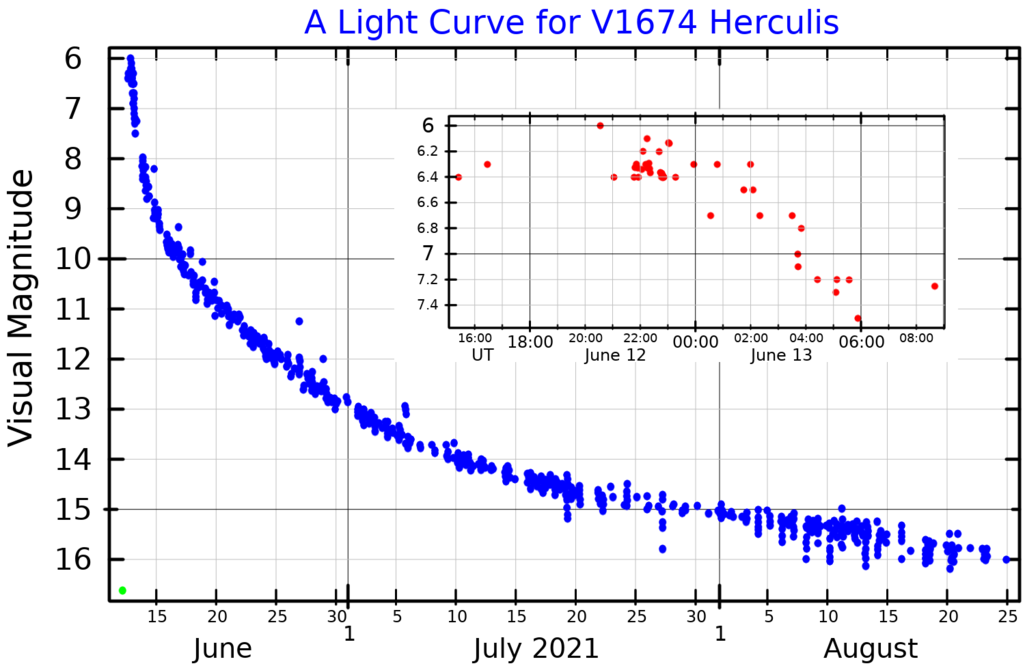
What we’ve found is that the fastest novae — i.e., the ones whose light falls off from its peak brightness the fastest — correspond to white dwarfs that are all extremely close to the maximum possible theoretical mass a white dwarf can have. The most massive white dwarfs whose masses have been determine through this method come in at about 1.37-1.38 solar masses: very close to that theoretical limit.
Many of these fast novae are, in fact, the white dwarfs associated with recurrent novae, such as RS Ophiuchi, and pretty much all of them have tight binary companions whose periods are measured in mere days.
But we also have the capability of measuring the light in many different wavelengths coming from these novae: the light from hydrogen; the X-ray light; the light that reveals the presence of specific elements, such as neon; and the abundance of the light elements and their isotopes that are very rare in the Universe overall, such as lithium-7. One of the great things about physics (and, by extension, astrophysics), is that every specific model you can imagine makes concrete predictions for what we should expect for each of these observables. The “shell” model of white dwarf accretion and novae, unfortunately, doesn’t agree with any of the things that we see.
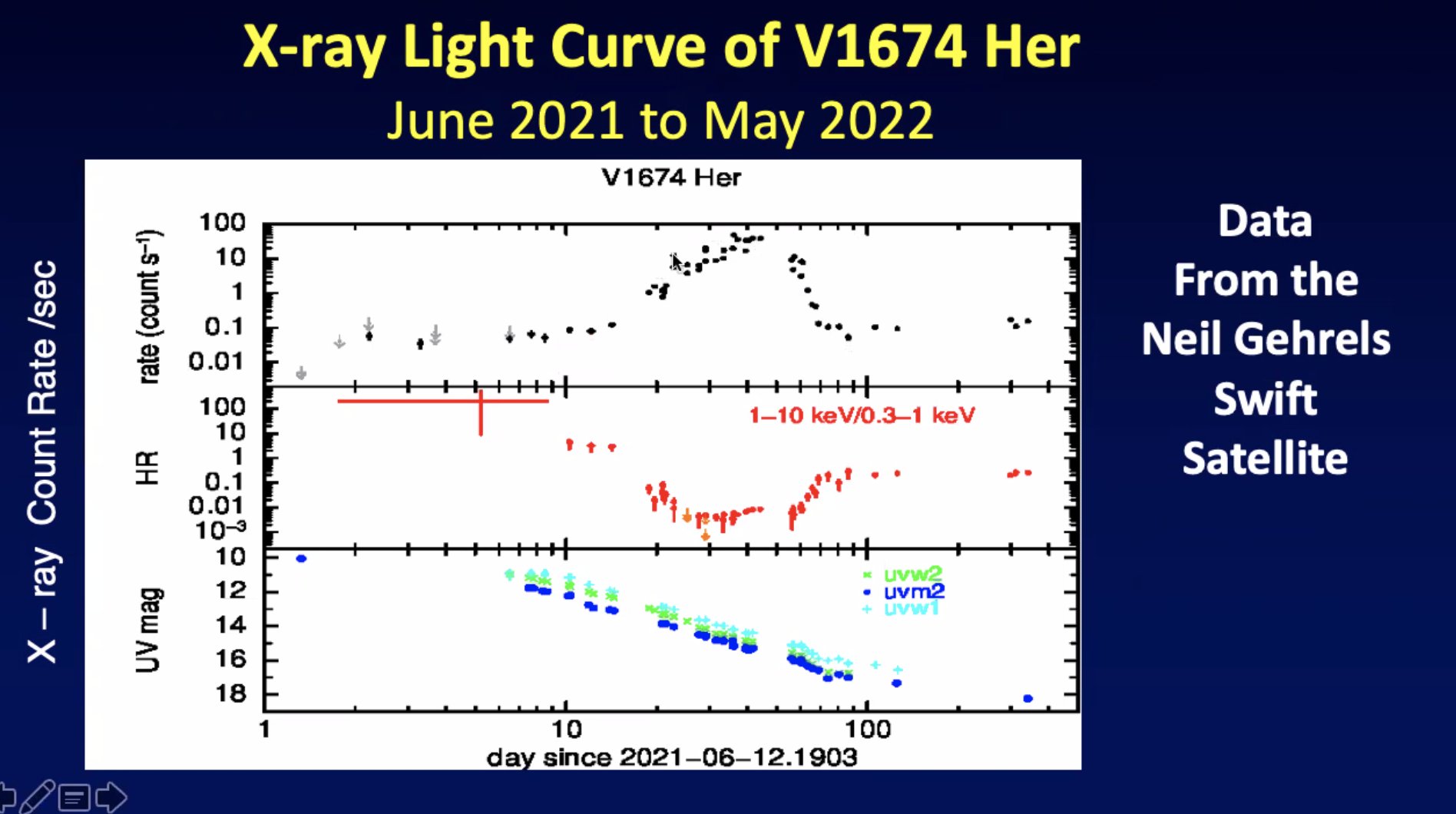
In fact, we’ve just observed the fastest nova ever recorded: V1674 Hercules, which occurred between a white dwarf and a very tightly-orbiting star located 15,000 light-years away from us. The light from the nova declined extraordinarily quickly: falling by +2 astronomical magnitudes (about a factor of 6) after just 2 days, and falling by a total of +3 astronomical magnitudes (a factor of ~16) in only 3 days. Its binary companion completes an orbit in just 3.67 days: 24 times faster than Mercury orbits the Sun.
When we examined the light from this nova, including the light from the ejecta, we found:
- the light emitted all over the electromagnetic spectrum rose to a peak and fell off very quickly,
- including the light emitted from hydrogen, which was likely siphoned off of the companion star,
- the spectrum of the nova contained neon, indicating that material from the nova was mixed with the white dwarf’s core,
- the gas was ejected incredibly rapidly: at speeds exceeding 6000 km/s, or more than 2% the speed of light,
- there were lots of X-rays emitted near the beginning of the nova, indicating that the eruption happened beneath the surface, and that X-rays “broke out” when the explosion reached the white dwarf’s surface,
- and lithium-7, one of the most easily-destroyed isotopes in the Universe, was found in a much greater-than-normal abundance, indicating the fusion of tritium (hydrogen-3) with helium-4.
The combination of these observations doesn’t agree with the initial, naive model of novae, but it agrees spectacularly with a model developed back in the 1970s and 1980s: the TNR model, also known thermonuclear runaway theory.
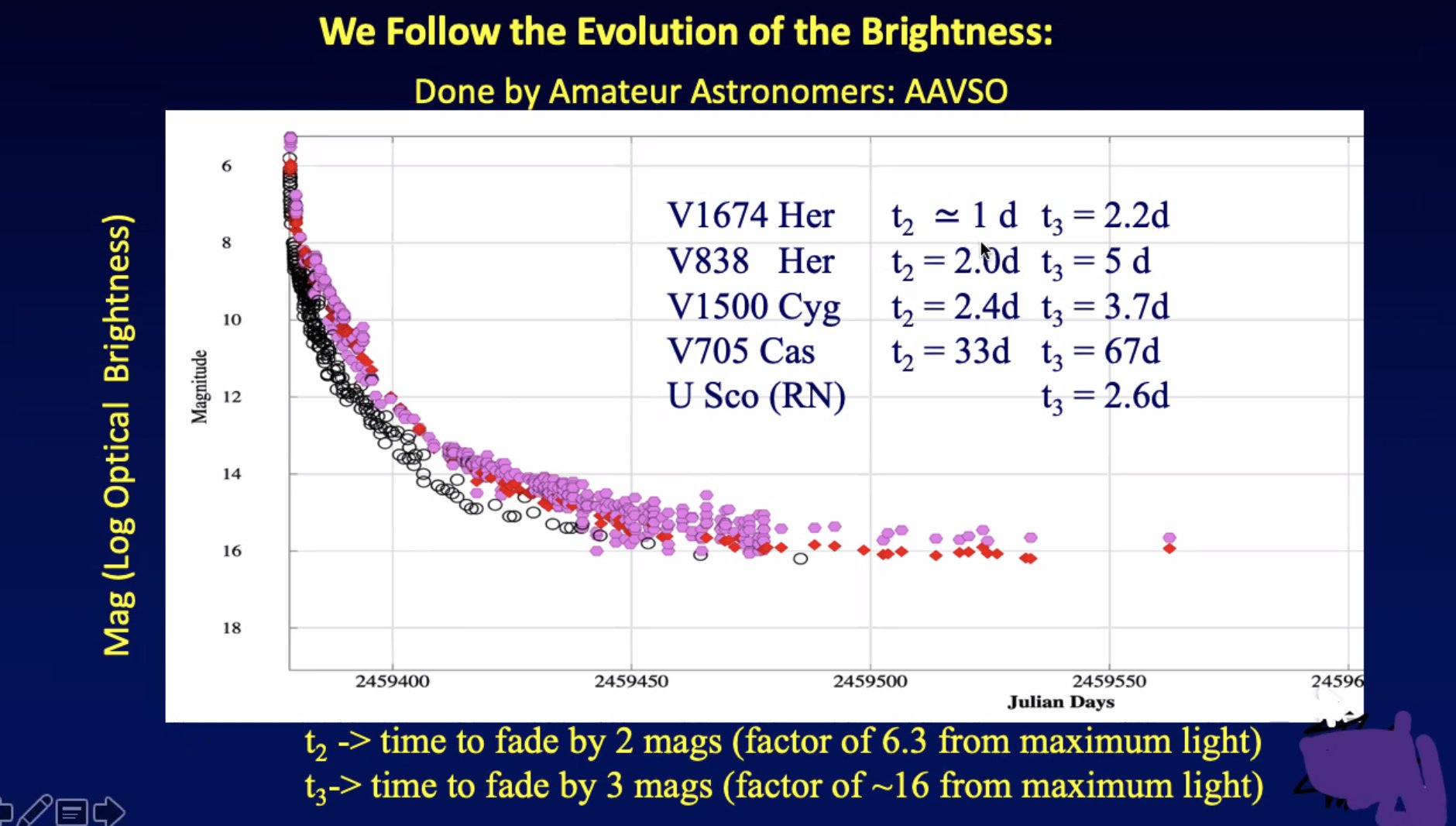
The idea behind thermonuclear runaway is that the matter accreted by a white dwarf from its binary companion doesn’t remain on the white dwarf’s surface, but rather mixes down into the white dwarf’s interior regions. The more massive your white dwarf is, the smaller it is in size, which translates to hotter and denser interiors. As a result, you need to accumulate smaller amounts of hydrogen — i.e., material that’s easy to achieve nuclear fusion with — in the white dwarf’s interior in order to trigger a fusion reaction and, as a result, an outburst that results in the phenomenon of a nova.
For less massive white dwarfs, you have to build up more and more material before such a fusion reaction can occur. Then, when that reaction does occur, because there’s so much material that can be fused, you get the ejection of a lot of mass, and the luminous outburst declines only very slowly.
On the other hand, if your white dwarf is more massive, you only accrete a small amount of gas before the nuclear reaction is triggered. The small size of the white dwarf leads to high peak temperatures, allowing for the fusion of heavier elements, high ejection speeds, and high-energy photons moving into the ultraviolet and the X-ray. Any obscuring, neutral matter gets ionized rapidly under these conditions, allowing us to see deeper into the outflowing gas very quickly.
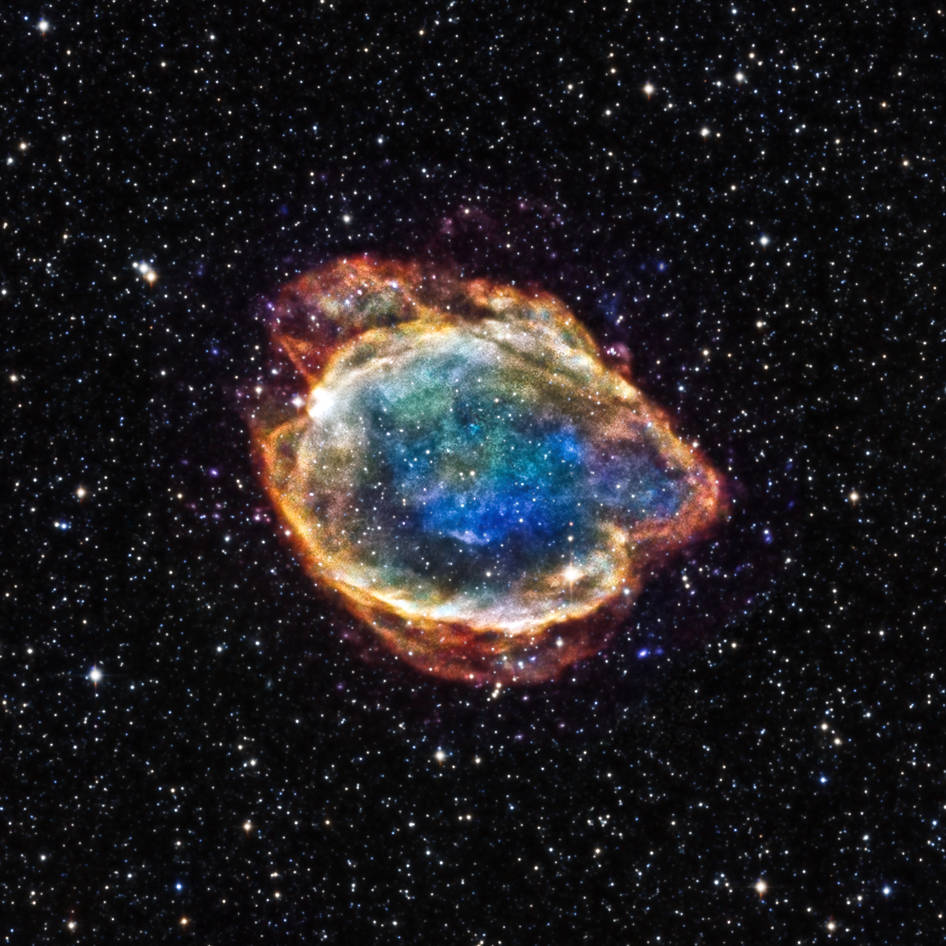
All of these predicted signatures of the thermonuclear runaway model were calculated, from a theoretical perspective back in the 1970s and 1980s. Although a few of them — like the presence of enriched light elements — were confirmed within only a few years of when they were made, most of this theory’s predictions were only observationally confirmed in the 2010s and, more recently, in the 2020s.
This model leads to one more spectacular prediction that’s never yet been confirmed: as white dwarfs get closer and closer to that ultimate mass limit, and to an eventual type Ia supernova, we expect the time between subsequent recurrent novae will get shorter, and the decline from peak brightness of each nova will get faster and faster. This gives us the triple expectations that:
- someday, one of these recurrent novae will someday go supernova,
- that V1674 Hercules should recur as well,
- and that there ought to be novae out there that brighten and decline so quickly that our current generation of telescopes may be missing them.
If nature is kind to us, all three of these predictions could soon be borne out.

Yes, of course it’s fantastic whenever we break a cosmic record. Finding the fastest nova ever — one that declines from its peak brightness faster than any other ever has — is exciting, but it’s simply representative of incremental progress in this field. One of the cutting-edge fields of astronomy is known as time-domain astronomy, which studies how objects in the Universe vary in properties (like brightness) over time. The faster we can survey large areas of the sky, and monitor how the objects within those areas change over time, the better we can get at finding and identifying objects that burst, flare, or even undergo runaway thermonuclear reactions on shorter and shorter timescales.
As long as white dwarfs keep accreting matter from a companion star, their march toward the maximum stable mass they can attain, the Chandrasekhar mass limit, is inevitable. The closer they get, the faster their novae will be, and the shorter the recurrence time will be between subsequent novae. If we’re smart, we’ll keep an eye on the fastest novae ever found to not only monitor when and how they recur, but to monitor them as potential supernova candidates. If nature is kind to us, we might soon witness the cataclysmic, supernova-producing death of a white dwarf remnant within our own Milky Way. With V1674 Hercules setting the new record for fastest nova ever recorded, we now have a new idea of where might be the smartest place of all to look.
This article was reprinted with permission of Big Think, where it was originally published.